This interview was originally published in Italian on Media Inaf, by Claudia Mignone:
To find out more about the potential risks to human health posed by the Van Allen belts and similar environments in space, Media Inaf talked to Piers Jiggens, an engineer from the European Space Agency’s Space Environment and Effects section, and a member of ESA’s Heliophysics Working Group, based at ESA’s technical heart ESTEC in the Netherlands. Originally from the UK, Piers graduated in aeronautics and earned a PhD in astronautics specialising in solar particle radiation and spacecraft design related to the threat posed from solar flares and solar eruptions.
Dr Jiggens, what are the van Allen radiation belts?
The Van Allen radiation belts are made of trapped “particle radiation”, predominantly protons and electrons. The Earth’s magnetic field, generated by the Earth’s iron core, is responsible for this trapping, binding them to gyrate around these magnetic field lines, and they bounce at low altitude when the magnetic field becomes stronger.
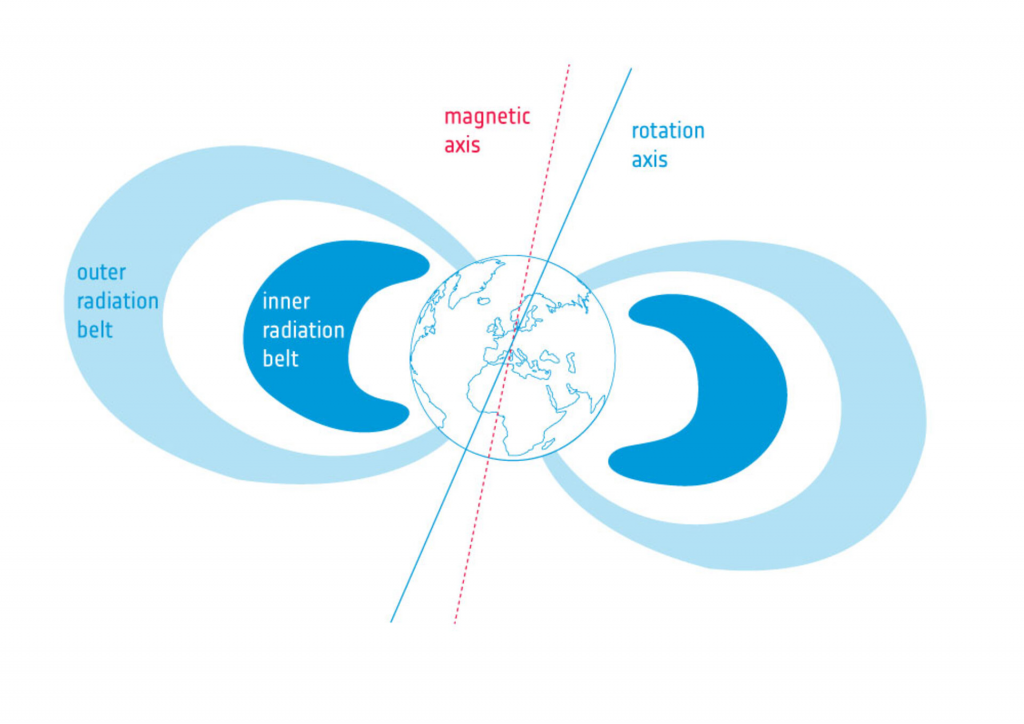
Where do these particles come from?
We normally consider there to be two belts, even though there is some filling of the gap between the belts at different times. The inner belt is largely made of protons and their origin is understood to be galactic cosmic rays from supernova explosions: these cosmic rays permeate the interstellar medium and a significant fraction can penetrate into the Solar System and Earth’s magnetosphere. When they impact Earth’s atmosphere, they release what we call “albedo” neutrons, which decay into protons and these protons are then trapped. The outer belt, on the other hand, is largely made of electrons and their origin is understood to be solar wind particles which enter the magnetosphere from downstream, behind Earth, via the magnetotail. When these are accelerated locally, within the magnetosphere, as result of geomagnetic storms, these particles are responsible for aurorae, which are one of the most visible aspects of space weather.
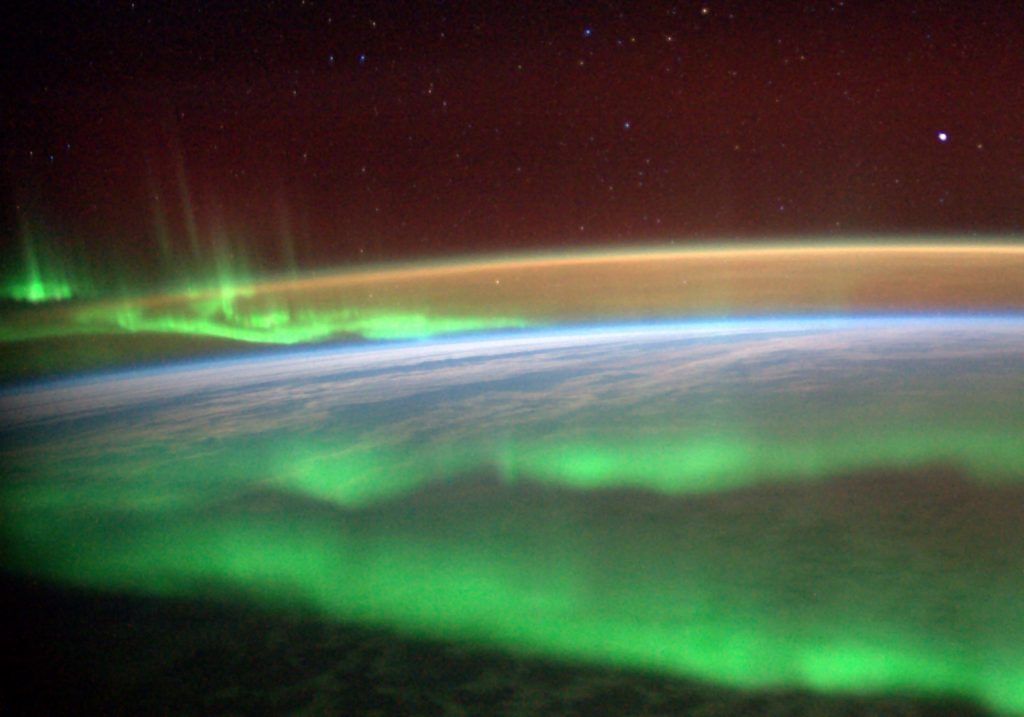
How dense is the environment we are talking about?
The density of particles of these energies, what we call particle radiation, is much lower than the plasma density of the plasmasphere which in turn is much lower than the neutral density within the Earth’s atmosphere. It depends strongly on location but let’s say something around 100 particles per cubic square metre in the radiation belts
Are there any risks for humans passing through the van Allen radiation belts?
There are mainly two types of radiation risks to humans. Firstly, what we call deterministic effects, which can be long term low-level, or short-term high-level radiation exposure: they result in disruption to the central nervous system, suppression of haematopoiesis in bone marrow, cataracts and other vision impairment, and acute radiation sickness, which would be a significant risk on extravehicular activities (EVAs, or spacewalks). Secondly, there are stochastic effects, which are normally thought of as an increased chance of cancer. Especially in the case of stochastic effects, heavier particles present greater risk of impact on human biology, because those heavier particles have a greater ionisation over a short distance. But it’s not necessarily the Van Allen belts that pose the greatest risk to humans, but indeed especially in the inner belt, there is some risk there.
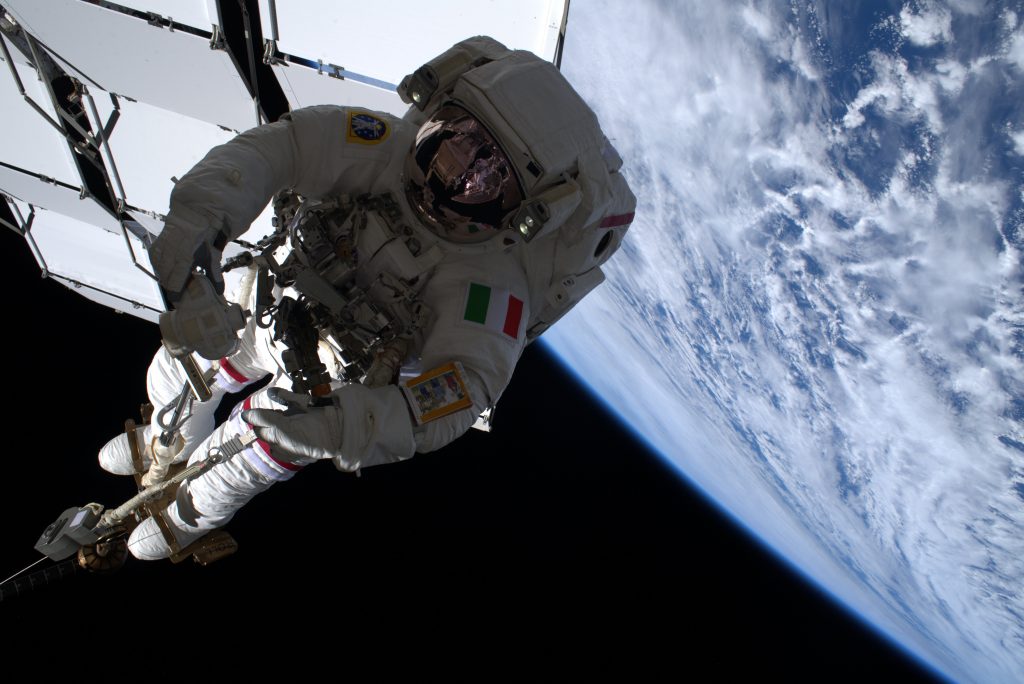
So they do not represent such a serious issue. Why?
Because we know where they are, we know when we’re going to fly through them, we can do that quickly and we know we can protect against them through material shielding and by keeping astronauts inside the spacecraft. It would be a very poorly planned mission if it suffered seriously from the Van Allen belts in terms of human health.
What poses a greater risk, then?
The galactic cosmic rays represent a greater risk because we know we can’t protect against them. Solar radiation storms also pose a more challenging risk because these are not easily predicted and they affect all of geospace with increasing severity, the further one is from the protective shield of the Earth’s magnetosphere.
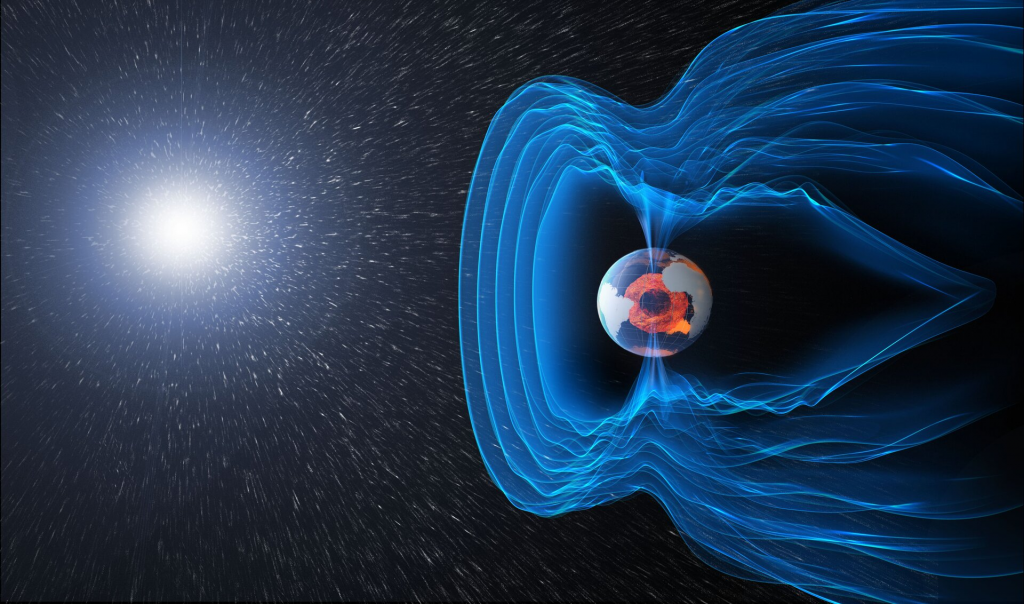
How many astronauts have already crossed the belts so far?
Technically any astronaut on the International Space Station crosses some parts of the belts at a low altitude but all Apollo mission astronauts will have flown through the radiation belts on their way to the Moon. The Apollo missions followed ballistic trajectories, so they passed through the belts very quickly which reduced the risk from this population to a very low level. Apollo missions took only about 4.5 days to get to the Moon. The Moon is just under 400 000 km away and the radiation belts only extend to around 60 000 km, so not a huge fraction of that time is necessarily spent in those belts. In terms of numbers, there were 9 Apollo missions transiting the entire belts (Apollo 8 and 10 to 17, Apollo 9 was low-Earth orbit only) and three astronauts actually made that journey twice (Jim Lovell on Apollo 8 and 13, John Young on Apollo 10 and 16 and Eugene Cernan on Apollo 10 and 17). So altogether 25 individuals – an exclusive club which will only be expanded now 50 years later.
Did these astronauts report any radiation-related symptoms?
That’s hard to say because there’s a lot of personal data that is protected. Some astronauts have reported “seeing” radiation – some kind of “snow” or flashes of light – that they can see even when their eyes are closed. This effect is well reported. The more long-term effects are harder to quantify because, if someone gets cancer, there is no definite way to say whether that has come from the radiation in space or through some other behaviour. So we try to think of it in terms of mitigating the additional risk from radiation.
How long can humans remain in such a particle radiation environment before it becomes dangerous?
Astronauts have survived in low-Earth orbit for long duration missions, several months up to years, especially when you accumulate time over various missions for a single astronaut, but the International Space Station provides a good deal of shielding. They regularly pass through the Van Allen belts, especially the inner belt, and through a region known as the South Atlantic Anomaly which is present due to the offset of the Earth’s magnetic field relative to the centre of mass. The magnetic field here is slightly weaker and those protons can reach farther down to the altitudes the Space Station transits, however this is not the harshest part of the belt. Crucially, particle radiation coming from outside the magnetosphere – from solar and galactic origins – are heavily attenuated by the Earth’s magnetosphere. This will not be the case for the upcoming Artemis missions, including the lunar Gateway. How long someone can remain in such environments depends a lot on the shielding afforded, which location you are in and the level of risk you are willing to take. Indeed survival itself is open to interpretation and increased risk of death over the lifetime of the astronaut is aimed to be kept at a very low probability. In this context there are fixed limits for astronauts and the role of space mission designers and operators is to keep the astronauts below the mission and short-term limits.
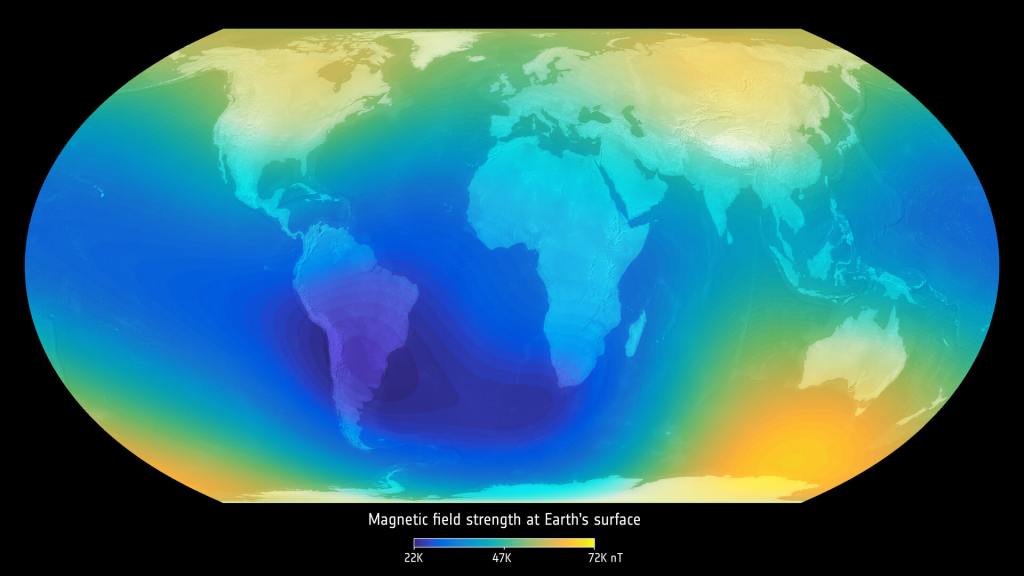
You mentioned shielding from radiation: how does it work?
If you know when you’re going to pass through the belts, for example, you can make sure to stay in a heavily shielded part of the Space Station. As for Galactic cosmic rays, they have such high energy that actually the shielding is quite ineffective, so you know that you’re going to accumulate a certain dose over an amount of time: that limits the duration of missions outside the Earth’s magnetosphere to about 500 days, which would be around about the time it takes to get to Mars and back.
Is there any way to get around this 500-day limit?
This is a little bit outside my domain, but how that radiation affects human biology is one of the critical topics for space exploration. There is still a lot of uncertainty, it’s an area that needs more research: otherwise you have to put additional safety margins and then maybe make a certain mission impossible. So if you can understand it a bit better, reduce those uncertainties, then maybe it will facilitate more missions. It’s an area of ongoing active research that I expect to get increasing focus as we go back to the Moon in this decade and then hopefully to Mars in the following decade.
What about materials: which ones make the most effective shield?
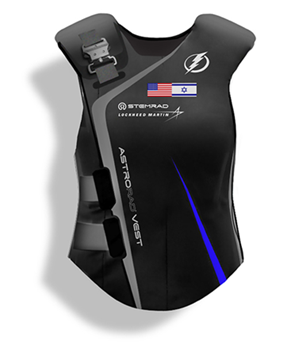
The biggest contribution for shielding is simply what you have up there, so what the structures are made of, which is a lot of aluminium. In the case of human space flight, they will also have all sorts of other equipment such as space suits. But there is a problem: when high-energy heavy particles impact shielding material, they produce fragments which can be more challenging to stop than the initial particle. This means that, in some cases, increased shielding actually increases the radiation dose: so at some shielding depth you have a sort of minimum, then with more shielding you start to see an increased dose due to these secondary particles. This is most relevant for galactic cosmic rays. To mitigate that risk, what we look for is shielding materials which have a low atomic number because in general they have a better fragmentation behaviour. Aluminium for example doesn’t have a very good fragmentation behaviour: other materials are better, such as plastics, polyethylene, or water. Water is obviously a good one for human space flight because you’re going to need to have some anyway. More exotic shielding materials such as those based on lithium have been identified as better still. The problem with lithium-based composites is that they are chemically unstable but work is underway to explore viable materials. In general, the strategy is either multi-functional materials (water, food, waste), optimised accommodation (e.g. accumulating traditional plastic shielding and multi-functional materials around a very sheltered part of the spacecraft) and innovative materials such as those based on Lithium.
Did they deal with this issue differently at the time of the Apollo missions?
For the radiation belts they stayed inside during transit and flew through them very quickly. There was a large solar storm that occurred in August 1972 which fortunately was between the Apollo 16 and Apollo 17 missions but it’s postulated that this could have posed a severe risk especially for astronauts on EVA on the lunar surface. There was no strategy to mitigate this risk at that time (as far as I know).
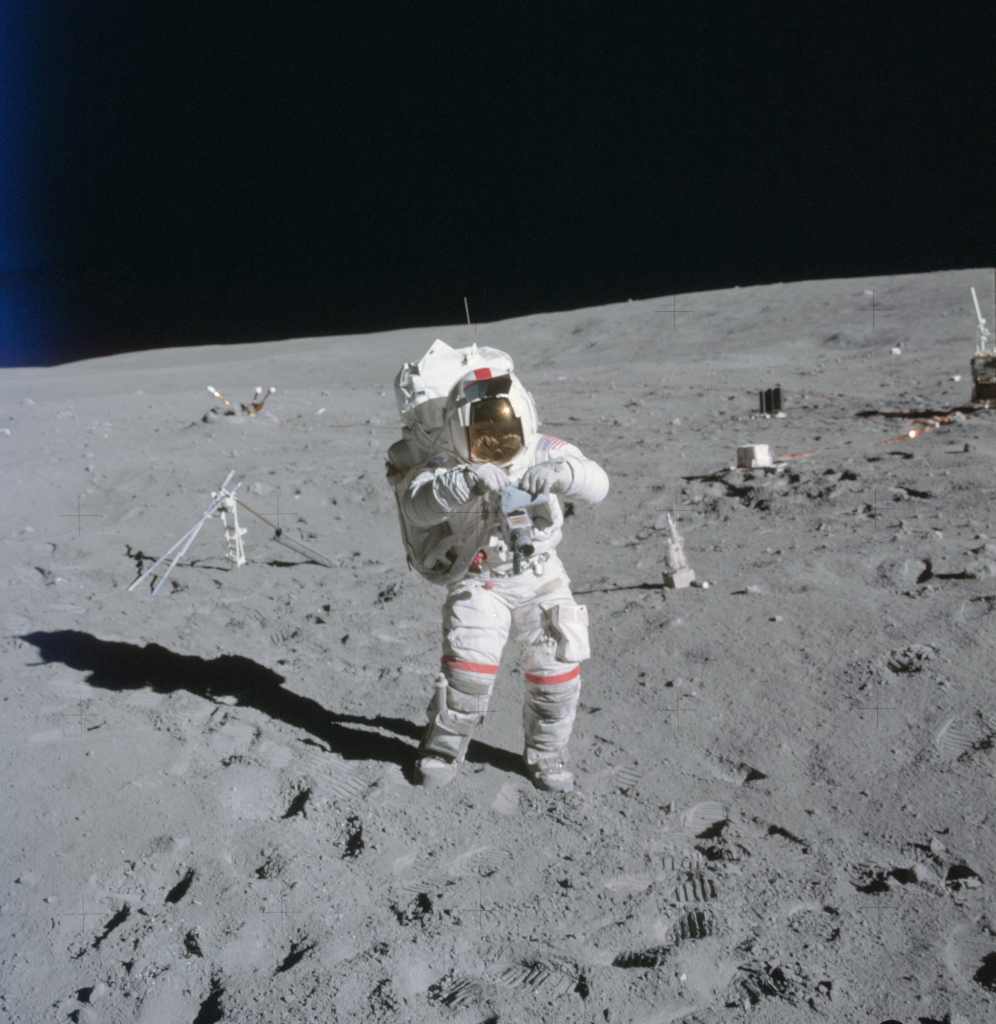
What about the strategy for future missions to the Moon?
For obvious reasons, astronauts on a lunar mission will fly very quickly through a radiation environment such as the van Allen radiation belts, but that’s not a very efficient way of getting to the Moon, for example. There will be a lot of systems, like electric propulsion systems, where you need to lift a bigger mass and it takes longer to get there. As part of the first elements to launch to the upcoming lunar Gateway, the Power and Propulsion Element (PPE) and the Habitat and Logistics Outpost (HALO), which are foreseen to fly no earlier than November 2024, ESA is delivering the European Space Radiation Array (ERSA) and Internal Dosimeter Array (IDA) payloads in collaboration with JAXA and complementing the HERMES (Heliophysics Environmental and Radiation Measurement Experiment Suite) payload from NASA. These will be a great addition to radiation belt observations during transit, as it will take over a year for these elements to traverse the belts and arrive to the lunar orbit, and, once on orbit, will give us the capability to nowcast the environment to give warnings to astronauts. In the future we hope to deploy a more capable suite of instruments to fully meet the needs of human space exploration. This would complement other missions imaging the Sun and the solar corona to provide forecast capability. It’s going to be an interesting time, the next part of this decade, and especially the next decade, for exploration.
You mentioned earlier the risk from radiation connected to solar storms, which are rather unpredictable. How can that be mitigated?
We look to minimise the effect of these particles through mission design, however, for operations it becomes increasingly important that we monitor space weather and develop capabilities to forecast solar radiation storms and give warnings to astronauts. If astronauts retreat to storm shelters (heavily protected areas) inside the spacecraft the solar radiation storm risk is largely mitigated, they might even surround themselves with extra material to improve the shielding. But if they are on EVA when these storms occur they could be at risk from effects which could endanger them and the mission pushing astronauts over their radiation dose limits or giving rise to short term effects endangering their safety.
Is there a best time in a solar cycle to plan such missions?
Interestingly, the best time to launch a long duration human exploration mission is during the solar maximum when solar flares and interplanetary coronal mass ejections are more frequent and more dangerous. This is because the background level of radiation, coming from the galactic cosmic rays, varies over the solar cycle and is lessened by a more active Sun.
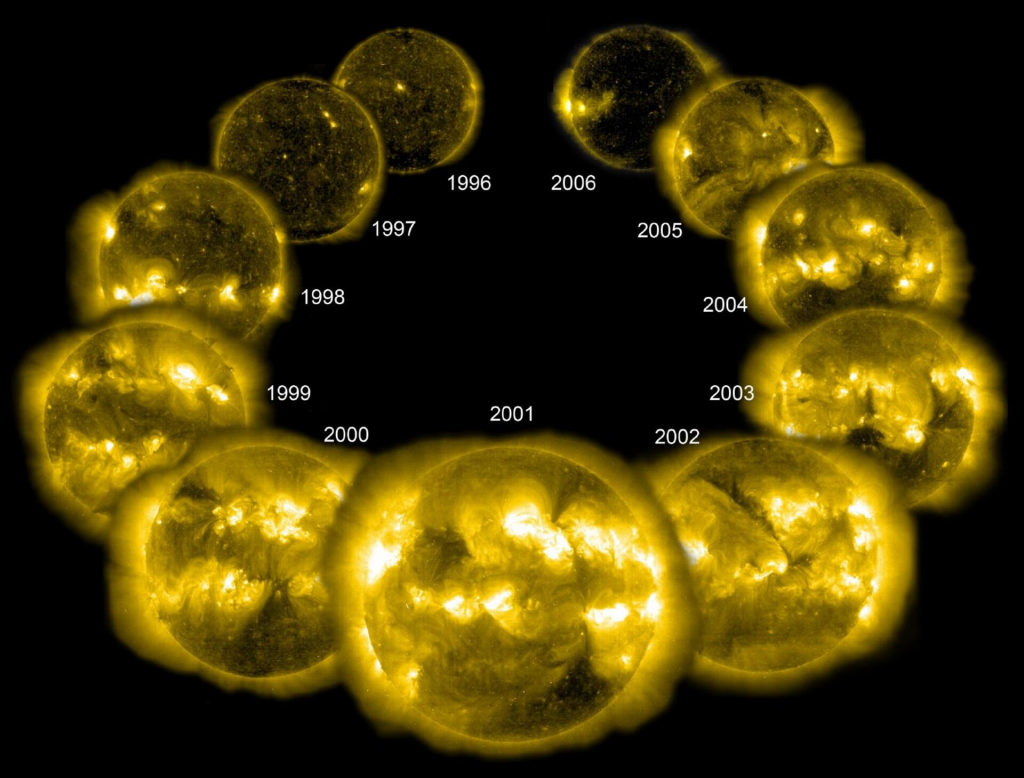
This is slightly counter-intuitive…
Yes and the reason is we can’t stop the galactic cosmic rays, they’re too high energy and you’re going to suffer that dose, so the only way to reduce it is to fly when the Sun is at its strongest, deflecting more of these particles as a result. Of course, if you fly during a solar maximum it becomes even more important that you can predict these radiation storms, which is nice for those of us that work in space weather because it makes it a more important field to study for the effects of human space flight. It’s a significant challenge because some of these storms happen very quickly: we talk about having to try to predict the eruption on the Sun, not just the effect that eruption would have.
Does the radiation environment extend all the way to the Moon? How do the risks change from International Space Station to Gateway missions?
Around Earth we have we have a lot of protection: if you fly over the poles, you would be open to high-energy particles coming from the outside, but the Space Station has an inclination of 51-52 degrees so it means that you’re not really flying over the poles and so you don’t see that that radiation, or at least you don’t see the full the full force of it. So in low-earth orbit you probably want to avoid being on an EVA when you pass through the proton van Allen belt, but it’s relatively easy to deal with. Also, the International Space Station is a big space station with a lot of material, so you’re kind of pretty well protected. Once we are beyond the inner magnetosphere, the radiation belts drop to a low level but the galactic and solar populations are un-attenuated. We cannot do much about the galactic populations during operations and it’s a relatively predictable slowly varying flux.
What about a journey to Mars scenario?
As we look to spend more time in deep space, we need to model in detail the radiation environment inside the human capsules and gateway. The duration of these missions in deep space will be much, much longer than those that astronauts have been on before, and that means your total radiation dose level can get quite high. As I mentioned, the galactic cosmic ray population is thought to put a limit on the duration of mission in deep space to keep below radiation limits when we factor in the uncertainties of the radiobiological effects to something like 500 days. This means more research is needed into these effects to satisfy mission planners that the risk to astronauts is acceptable.
The main difference between the Moon and Mars, I think, is that Mars missions will have to be much longer because you have to wait for the alignment of the planets to return so that means you’re already in the kind of 500-day range. Lunar missions can be much shorter and if someone suffers some radiation exposure, they could possibly return sooner. Also, the infrastructure we have around the Earth for forecasting solar storms and measure the radiation, they can be used for the Moon but for Mars we’ll need a different set of infrastructure. It’s interesting to note that the eruptions on the Sun that could impact Mars most acutely can be on the part of the Sun you can’t even see from Mars, they’re beyond what we call the West limb, whereas in Earth it’s about 60 degrees to the West Side, the right side of the Sun, where the best transport conditions exist for the radiation.
Are there any medical devices that astronauts could operate in the case of radiation induced sickness on a long-term mission, for example to Mars?
If you mean some Star Trek-like jab which helps you with radiation, to my knowledge it doesn’t really exist. I think the most important thing would be to help them to get back inside and get out of their spacesuit, because if you really become physically sick due to radiation, the worst thing would be to be inside a contained suit. You probably will have other problems but you don’t want someone throwing up inside a microgravity environment. I think just helping them inside would be the main assistance that others could give, and it would be a significant benefit of having more people there on the spacecraft than just one or two.
Do Mars and other planets have something like the van Allen belts, too?
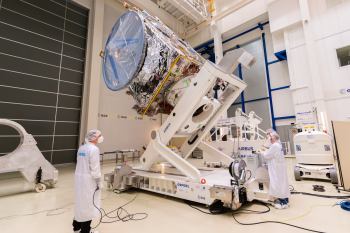
Yes, Jupiter has a very strong magnetic field and trapped radiation environment. It is said that if you could see Jupiter’s magnetosphere, it would appear from Earth as large as the Moon. It’s a very challenging environment for space missions like the upcoming ESA Jupiter Icy Moons Explorer, Juice, which has a dedicated lead vault to house components for extra protection. Other gas giants also have radiation belts whilst planets like Mars and Mercury have only very weak, relic fields and where only transient trapping of particles occurs: they’re normally very low energy and not of great concern.
The particle radiation environment is also a problem for uncrewed space missions. What happens to electronics: what are risks from radiation that affect spacecraft components?
There are four different types of radiation effects on spacecraft components: ionising dose effects, which means that the particle radiation is depositing energy in a sensitive area and it can over time change its properties; non-ionising dose effects, which are normally displacements of the lattice structure and can also change the properties of the target; single-event effects, which are more short term, they could be anything from a false signal to just a memory upset or something more critical, which leads to a destructive feedback loop; and finally internal charging, which is caused by high energy electrons where an amount of charge is deposited in a component deep inside a spacecraft and it’s too well isolated so that builds up and then you have a discharge.
How do you mitigate these effects?
A lot of it is done through the design of the components. It can mean that the space components can be a bit heavier, a bit more power hungry. There’s a lot of testing that goes on as well: that’s the most important thing when you’re going to fly a component, you don’t just test one processor but you test all processors. That, of course, is combined with our understanding of the environment. We don’t suffer loss of missions from radiation very often and it would be bad if we did.
Discussion: 2 comments
I’ve read, that the sun is entering a phase of higher activity now. I’ve always thought, that this was bad luck for manned Artemis missions, but it sounds like an advantage in this article. Do you happen to know how this knowledge has been gathered? Has it been through mesurements on the ISS?
ESA has space weather measurements in place, and an actual space weather office: https://www.esa.int/Space_Safety/Space_Weather_Office