From the earliest clear views of comet 67P/Churyumov-Gerasimenko it was obvious that the surface is a collection of contrasts: smooth plains, imposing craggy cliffs and scatterings of boulders. Rosetta scientists are now digging into the detail to explain how some of these features may have arisen and what this means for our understanding of comets.
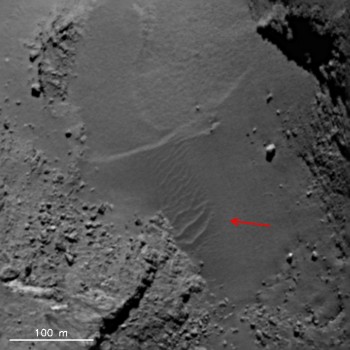
Ripples in the Hapi (neck) region are attributed to a phenomenon known as airfall.
Image credit: see below.
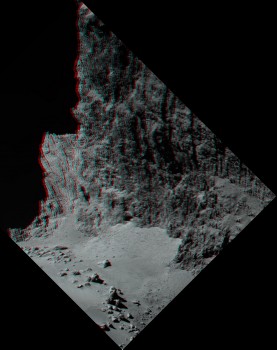
Boulder field in Hapi region. (Individual frames used for the anaglyph can be found below.) Image credit: see below.
The study of comet 67P/C-G reveals a dramatic surface environment where considerable amounts of material – up to 1000 kg per second – are ejected from the comet. Not all of this makes it into space, instead some falls back to coat the nucleus.
These small, solid particles – typically with sizes ranging from micrometres to tens of centimetres – are ejected when icy material sublimes. As the ice turns from solid to gas, it escapes into space, propelling the solid particles with it. The smallest of these expelled dust grains – millimeter-sized or smaller – obtain sufficient velocity to escape the influence of the comet and become part of the comet’s tail, which can stretch for millions of kilometres through space.
But some of the larger particles (centimetre-sized or greater) fall back, meaning that particles from one part of the comet can descend to the surface on another part of the comet’s double-lobed nucleus. This ‘airfall’ creates smooth plains that can be as much as a few metres thick.
Nicolas Thomas from Universität Bern, Switzerland, and collaborators have used data from OSIRIS, the science camera on Rosetta, to study these dusty plains. They then used computer models to investigate the mechanisms at work.
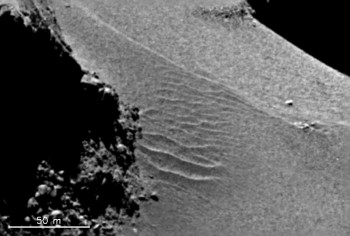
Close-up of the region in Hapi indicated by the red arrow in the image above. The ripples are attributed to a phenomenon known as airfall. Image credit: see below.
The neck, where the two lobes join together and which has been named the Hapi region, has been notably active and so provided an obvious site for investigation. Computer models show that particles ejected from Hapi with speeds below 0.8 m/s will become airfall.
Within the neck, there are ripples in the dust that resemble wind-blown features on Earth and other planets. To understand how these are produced, the team modeled the gas dynamics near the surface of the comet. They found that escaping gas could quickly reach 500 m/s.
Although this speed would partly compensate for the extremely low gas density around the comet, the escaping gas would need to be located very close to the dust deposits to allow the ripples to form. This led the team to investigate other possibilities.
On Earth, the major force to overcome when sculpting wind-blown ripples is gravity holding the grains in place. On the comet, where gravity is minuscule, the major hurdle is the cohesive forces between the dust grains holding them together. This realisation opened another possible formation scenario.
A second computer model showed that as the airfall hits the surface, it dislodges the blanket of particles already there. This makes them more susceptible to weaker gas forces, and also allows the comet’s feeble gravitational field to pull them into the linear features seen in the images.
Although there are many details that remain to be calculated, it is now clear that the airfall phenomenon is an important process in defining the surface properties of a significant fraction of 67P’s nucleus.
Dusty plains are not the only thing covering the surface of the comet. There are also boulders scattered across the dramatic landscape. Maurizio Pajola, University of Padova, Italy, and colleagues have identified 3546 boulders larger than 7 metres in size.
They studied OSIRIS images taken on 5 and 6 August 2014, when Rosetta was close enough to resolve 2.44-2.03 metres per pixel. On an imaged surface of 36.4 km^2 – this is 75% of the total surface of 67P – the number of boulders larger than 7 metres averages to about 100/km^2. Knowing what the boulder distribution was on most of the comet’s surface was a crucial input, provided by Maurizio and by Jean-Baptiste Vincent, to the landing site selection process last year. Since then, Maurizio and his colleagues have been able to study the boulders and their distribution in more detail.
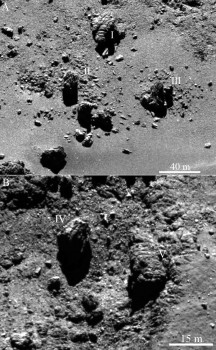
Large, fractured boulders in the Imhotep region, surrounded by material that appears to have split from the boulders. Image credit: see below.
These boulders are not uniformly distributed across the comet. On the smaller lobe, often dubbed the head, there are more smaller boulders than on the larger lobe, called the body. In particular, the size distributions are related to how fractured the formation area is. Thermal stress causes fractures on the surface, which can dislodge blocks – forming new boulders – and there is also a continuous fragmentation of boulders that have already been formed. By examining the frequency with which boulders greater than 7 metres are found on the head, the neck and the body of the comet, the team shows that the head is more fractured than the body: the size-distribution for the head is steeper than that of the body.
In the neck region, there is more uniformity between the number of large and small boulders. One particularly intriguing boulder field (see anaglyph above) occurs here and the team suggests that it was created by blocks falling from the cliffs of Hathor, which are located on the body of the comet.
The team has identified three possible reasons why there are fewer small boulders in this field. It could be firstly, because small icy boulders have sublimed away; secondly, small icy boulders could have been ejected from the comet’s surface by outgassing events; thirdly, airfall could have covered the smallest boulders removing them from view. It could be any one or all three mechanisms at work simultaneously.
In all of this work, planetary scientists are starting to build a picture of the processes at work on 67P. It is clear that the comet is a place of sudden change and gradual evolution, making it all the more fascinating to watch as Rosetta’s mission continues.
This blog post is based on the papers “Redistribution of particles across the nucleus of comet 67P/Churyumov-Gerasimenko” by N. Thomas et al., and “Size-frequency distribution of boulders ≥ 7 m on comet 67P/Churyumov-Gerasimenko” by M. Pajola et al., both published in the Astronomy and Astrophysics special issue on Rosetta mission results pre-perihelion.
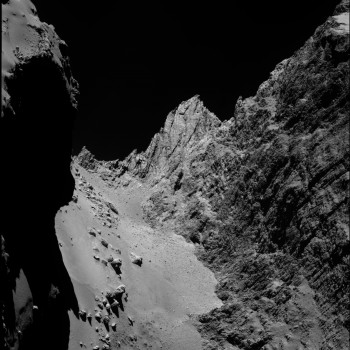
Boulder field in Hapi region. (One of two frames used for the anaglyph above. This is the left-eye view.) Image credit: see below.
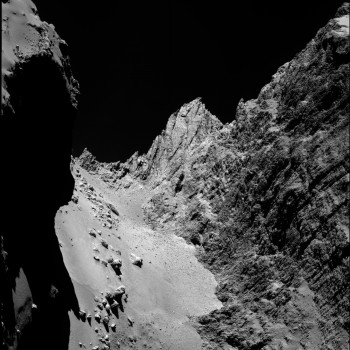
Boulder field in Hapi region. (One of two frames used for the anaglyph above. This is the right-eye view.) Image credit: see below.
Image credit for all images on this page:
Credit: ESA/Rosetta/MPS for OSIRIS Team MPS/UPD/LAM/IAA/SSO/INTA/UPM/DASP/IDA
Discussion: 30 comments
I haven’t read the papers yet, just this blog post.
The boulders depicted on Imhotep are air fall too.
I note that this post refers to ejection and return of air fall, saying that it consists of solid particles “typically with sizes ranging from micrometers to tens of centimetres”. So the air fall is not considered to include the 7-metre-plus boulders studied by Maurizio Pajola and colleagues.
It is clear therefore (from this blog post at least) that Pajola et al are referring to large boulders that are related to their surrounding fractured “formation area” and have broken away from that formation area due to thermal stress. These boulders are then said to be subjected to further erosion: “and there is also a continuous fragmentation of boulders that have already been formed”. Presumably this fragmentation is a continuation of the thermal stress erosion of the boulders, remaining in the formation area and never moving.
The photo supplied as evidence for this in situ fracturing from the formation area and ongoing fragmentation is of “large fractured boulders in the Imhotep region, surrounded by material that appears to have split from the boulders”.
I wonder if the authors noticed that the material that’s split from the boulders has a distribution that is biased westward along the rotation plane. This can only be explained by these boulders being air fall as well, orbiting in a suborbital trajectory backwards down the rotation plane, landing with an entirely westward velocity component. The scree riding on their backs would then continue for a few more metres before landing, explaining the westward distribution bias. And of course, some crumbling may have occurred on landing too, due to compression forces. Those fragments would also land a little westward.
These boulders on Imhotep are clearly not rocks that have been broken away from a supposed formation area in there immediate vicinity. They have arrived in a backwards trajectory down the rotation plane, landed and shed their material westwards, even further down the rotation plane. The most obvious example of one of these boulders is the 30 million kg boulder on Site A that drifted 170 metres back down the rotation plane, shedding its scree further westwards across the flat area of that crater. This is a very clear signature and the stretch blog post for it has been linked numerous times on this blog by me and Marco.
It is indeed intriguing that this gigantic boulder sitting marooned in the middle of Site A hasn’t been mentioned at all (in this Rosetta blog post). Surely, in a paper concerning itself with 7-metre-plus boulders fragmenting from their “formation area” and exhibiting further supposed thermal fragmentation this 170m x 30m x 30m isolated boulder would garner special attention. Could it be that it just cannot be explained, sitting as it is, in the middle of such a flat area?
Since all these boulders are air fall, they need an mechanistic explanation. It seems gas propulsion is off the cards according to the sizes given for air fall in this post. The only other explanation is ejection via ‘centrifugal’ force into a suborbital trajectory. This process has also been described exhaustively on the stretch blog: collateral detritus to the giant slabs that departed the comet on spin-up to fragmentation. Some boulders would by chance have received a negative delta V kick, drifted suborbitally backwards down the rotation plane, landed with a 100% westard velocity component and ejected scree etc further westwards.
That is what we see with this group on Imhotep. You can even see where they have come from- its the curved, sloping area to the east of the so-called roundish features where similar-sized boulders are embedded in the slope. If you draw a corridor the width of this area of exposed boulders and in line with the rotation plane, it will embrace this clutch of boulders as it is extended westwards across the flat plain of Imhotep. It takes in this clutch that’s cited along with another clutch nearby including Cheops. Areas outside this corridor are devoid of large boulders. The association couldn’t be more marked.
The area of embedded boulders was exposed by the departure of the Imhotep missing slab. Some boulders escaped the comet with the slab, some remained exposed, and the few we see constrained in the corridor are those that got the backwards delta V kick (with respect to prograde rotation).
Any word on when of if the OSIRIS data will be released?
–Bill
Hi A. Cooper,
re
“It is indeed intriguing that this gigantic boulder sitting marooned in the middle of Site A hasn’t been mentioned at all.”
That’s simply, because it isn’t a boulder, but a remnant left over from surface erosion.
Hi A.Cooper,
for larger boulders, “rock”fall/”icefall” is the easiest explanation. Rockfall happens every now and then on celestial objects with a solid surface. Why invoke unnecessary centrifugal force ad-hoc scenarios?
Since we finally had the huge opportunity to see one of the Osiris images, I checked the release status of the images of this instrument in the archive browser. I found the most recent Osiris images are 15-18months old approx: a time when the comet is just a white dot.
Then I checked in the blog and found this:
When will OSIRIS images and other data from the mission be available?
“The new image viewer is essentially a ‘beta release’ and is currently exclusively for NAVCAM images. However, it is planned to extend it to include data from other Rosetta instruments: the release of scientific data from these instruments covering the period January–November 2014 is foreseen starting in May 2015, depending on the instrument.”
We are in November 2015. What is the timeline to release Osiris images ? It seems the 6 month delay is not something that will happen. But I now feel we will never see these images.
Do we know what those expanses of dust are actually made of?
Here’s a picture to illustrate what I’m talking about in my comment above:
https://scute1133site.wordpress.com/a-provenance-for-cheops/
Gerald says:
09/11/2015 at 18:21
Hi A.Cooper,
for larger boulders, “rock”fall/”icefall” is the easiest explanation. Rockfall happens every now and then on celestial objects with a solid surface. Why invoke unnecessary centrifugal force ad-hoc scenarios?
I’m not sure I see the low gravity of 67P providing the energy to move anything that far from any of the areas that it might have come from.
Judy
Judy
I think you were right when you said all those months ago that you thought the Imhotep slab was more like a dome when it was seated. I was saying it was a single, thick, flat slab. However, since then I’ve seen photos from favourable views showing three torn strata, or onion layers around the edge. This suggests the slab was three strata layers thick. I don’t know if you still agree with my and Marco’s hypothesis of slab loss but your dome idea was rattling around somewhere in my head when I saw that photo. I don’t think it was domed like a pudding bowl but somewhat more domed than a flat slab. Please see my reply to Gerald, which is relevant to your point about whether the boulders eroded from the cliffs or not.
Hi Judy,
gravity and inertia of mass balance each other.
Gravity results in potential energy, and inertia in kinetic energy. To understand rockfalls in microgravity, it’s important to be aware of the inertia of mass.
A “rock”fall, as soon as the fragments are dislodged from the ground by thermic cycling or sublimation/erosion, runs almost like a rockfall on Earth, just in (very) slow motion.
Fragments in microgravity come to rest close to the location they would come to rest in Earth gravity.
The difference to a rockfall on Earth is mainly, that fragments of the same hardness would be crushed on Earth, whereas they may survive in microgravity.
For small grains, molecuar forces (van-der-Waals forces) play a more prominent role in microgravity than on Earth.
More formally a back-of-an-envelope calculation:
For mass m and local constant of gravity g, assume a fragment of mass m falling down the height h, then sliding horizotally with the starting with the same (kinetic) energy.
This means, potential energy
E = m g h
is transformed into kinetic energy, which then does work
W = F_k s
of the same amount of energy during horizontal sliding, meaning
E = W.
The force F_k of kinetic friction is
F_k = mu_k F_n,
with the weight as normal force on a horizontal plane
F_n = m g,
and the coefficient of kinetic friction mu_k.
Now set in the formulas for E and W:
mu_k m g s = mu_k F_n s = F_k s = W = E = m g h,
in short
mu_k m g s = m g h,
Divide by m g, both non-zero:
mu_k s = h,
This equation is independent of mass and gravity.
You may resolve it to
s = h / mu_k.
mu_k is usually a little below 1 for many materials. In a very dynamic situation, where fragments roll, instead of slide, the value may be well below 1.
Therefore it’s reasonable to assume fragments being displaced horizontally at least as far as they are displaced vertically, some (the roundest) possibly several times further.
https://en.wikipedia.org/wiki/Mass#Inertial_vs._gravitational_mass
https://en.wikipedia.org/wiki/Potential_energy#Local_approximation
https://en.wikipedia.org/wiki/Work_(physics)#Work_and_energy
https://en.wikipedia.org/wiki/Friction#Kinetic_friction
https://en.wikipedia.org/wiki/Normal_force
https://en.wikipedia.org/wiki/Weight#ISO_definition
… sorry for the typos…
I should add: Gravitational potential energy isn’t the only source of energy convertible to kinetic energy:
A velocity of a few centimeters per second are sufficient to displace fragments considerably, remember Philae’s landing.
These velocities may obtained due to gas pressures of as low as about 1 hPa in some settings, by sudden release of thermal stress, or by saltation, meaning by collision with moving fragments.
Gerald
I agree with your calculations. So that means that by and large we can look at these isolated boulders and the cliffs they came from and imagine whether they could have travelled that far if on the Earth. “By and large” would mean notwithstanding some small differences like air resistance.
I checked the pictures in scientific paper from the “Inside Imhotep” Rosetta blog post in July 2015. One shows gravitational heights above the lowest point on Imhotep, using colours. It appears the nearest cliff to Cheops is about 700 metres away and perhaps 150-180 metres high. That’s a 3.88 to 4.66 distance/height ratio. That’s probably at the upper limit of your estimates for rolling or sliding several times further than the cliff height. However, I think it’s doable since several of the boulders on Hapi have long tracks going back to the undercut cove (incidentally, these Hapi boulders have been misattributed to falling from Hathor, in the same paper).
Here’s the blog post for Inside Imhotep:
https://blogs.esa.int/rosetta/2015/07/20/inside-imhotep-2/
Here’s the paper linked in the blog post (still free to read; see fig. 4 for gravitational heights).
https://www.aanda.org/component/article/?access=doi&doi=10.1051/0004-6361/201525947
Despite doing my best to verify your possible rock rolling scenario there remain some strangely ad hoc traits that arise from it. One is that there is the distinct westward bias related to the fragmentation detritus of these rocks as they supposedly sat there eroding on the dust for millennia. What process could allow that westward bias to occur? A related issue is why the detritus is not just westward but spread up to 100 metres westward instead of being piled up at the base of the supposedly eroding boulders. Whilst I too would expect some ongoing erosion and even fragmentation, that process doesn’t explain the distribution of the related detritus that we see.
So the westward bias and the 100-metre fragment migration are two ad hoc issues to address. The third is the coincidence that none of your rolling/sliding rocks ended up outside the rotation plane corridor that runs from the embedded boulder field. It’s true that there are three large boulders much closer to those cliffs but they are of a different shape and character. They’re much more like the tight clump of three rocks on Aker, just round the corner from Imhotep because they have the same characteric rectangular look, one of them with the same longitudinal groove. And, interestingly enough, if you produce their rotation plane corridor round to Aker, it skims near to the other three rocks.
In fact, all the major features on Imhotep can be slotted into neat rotation plane corridors of two distinct types: 1) the boulders and their breccia (embedded boulder field) source and 2) the roundish features and the flat, boulder-free plain. I’ve already done a link above for the upper, northern boulder field and its corridor from the breccia field. As for the roundish features and the flat, dusty plain, it’s more to do with the absence of boulders around the roundish features giving rise to a completely boulder-free rotation plane corridor. That corridor runs westward from the roundish features and most exactly encompasses the flat, pristine area of pure dust.
The following picture from the Inside Imhotep blog post depicts this very well. It’s from the same paper but was actually inserted in the blog post. You can draw rotation plane corridors in your mind’s eye at 90° to the “north” arrow. They would all be parallel to the rotation plane which is the equator. You can draw a wide corridor encompassing the green roundish features and see it sweep right through the pristine part of the dust plain. Clumps of boulders kiss the corridor on both sides but barely encroach upon it.
This photo also shows the same boulder corridor as the one in my other link. It’s quite clear because the boulders are red. There’s one concentration sited above the big red circle towards the right. That’s the embedded boulder source. And there’s the concentration out on the upper middle part of the flat plain. Those two concentrations are in line with each other along their own corridor.
https://www.esa.int/spaceinimages/Images/2015/07/Mapping_Imhotep
I would say the reason there are no boulders around the roundish features is that they are buried. That’s because the roundish features and the flat areas around them are indicative of slurry emerging and swamping the boulders. They never had a chance to be loosened by the giant Imhotep slab uplift (my and Marco’s hypothesised Imhotep slab uplift). The reason the embedded boulders were loosened and driven backwards is that in this scenario, the slab hinged up at this point, as predicted by stretch theory, and ground them out.
Whatever the ins and outs regarding why there were no boulders among the roundish features, the pattern is as clear as day:
1) the boulder source field has a twin clump of the same width spread westwards down the rotation plane corridor.
2) the roundish features with no boulder source have no corresponding clump at all down the rotation plane.
Hi A.Cooper,
I’m hesitating to give a clear answer, since there are several possible effects of unknown extent.
– We’ve seen boulders ejected by outbursts. Up to which size can boulders be displaced significantly by these events?
– How fast do slopes erode away relative to the degradation of boulders? If boulders are rather resistant to erosion with respect to slopes, slopes may have existed closer to the boulders.
– How many erosion-resistant boulders are embedded in the comet? Some boulders may have just sit near the location they are now, the surrounding matrix eroded away.
I’d think, post-perihelion images will narrow down answers to these questions, and we’ll get a better overview, which boulders actually sit on strange locations, after considering these effects.
It shouldn’t take too long any more, until we get these data..
… when looking to the cliffs around the annotated area of this image
https://www.esa.int/var/esa/storage/images/esa_multimedia/images/2015/07/mapping_imhotep/15527692-1-eng-GB/Mapping_Imhotep.png
I see lots of clasts (boulders) embedded in the matrix, part of the surrounding matrix already eroded/weathered. Further erosion/weathering would free the clasts, which then would fall or slide down to the annotated field of boulders.
Notions:
https://en.wikipedia.org/wiki/Clastic_rock#Clastic_sediments
‘ Particle-O-Sphere’ also takes a significant role on ‘imprinting’ Coma with non-radial dynamics. As dangerously showed with ROSETTA’s kinetics, it fills the roll of actual ‘air-drag’ to Everything, from molecules to satellites.
It’s actually not probable -and was my former thinking- that most of this ‘air-drag’ and deflection could be happening at the ‘inner’, close gas envelope [500m]. Right now preferring a low mass, ‘puff’ material, ‘percolating’ kinetics, as for what is seen near surface.
Speculating that, once ‘airborne’, Dense Particulate goes on extreme ‘peeling’ and ‘effervescent’ erosion mode. Those ‘peels’ quickly dry to Superfine Dust and doesn’t leave coma.
[Nucleus’ [electric] field becoming more necessary to explain unperturbed, ‘collimated jetting’]
Hi Gerald, Re:
Gerald says:
09/11/2015 at 18:21
Hi A.Cooper,
for larger boulders, “rock”fall/”icefall” is the easiest explanation. Rockfall happens every now and then on celestial objects with a solid surface. Why invoke unnecessary centrifugal force ad-hoc scenarios?”
These scenarios are neither “unnecessary” nor “ad-hoc”
The scenarios are *dictated* by the requirements of stretch. To say it another way, we are not inventing a scenario of centrifugal balance rates of spin just to explain the boulder, but noting that the centrifugal rate of spin required for stretch is precisely that which shows us how these “rocks” got to be where they are from where they originate.
Interesting discussion, but I guess I’m confused when the terms “rock fall” and “boulders” are used. I assume they’re being used because that’s exactly what they look like and for ease of description. However, I also thought that they could not be actual rocks or boulders because this doesn’t fit into the mainstream belief system. So can someone please clarify as to what the exact composition of the “rocks” and “boulders” are?
“Rockfall” is just the geologic notion for similar processes on Earth and other rocky planets.
I’ve used it here to avoid invention of neologisms like “clathratefall”, “dryicefall”, “mix-of-dust-and-ices-fall”, “regolith-slide”, “refractory material-slide”, etc.
Regarding composition: Take carbonaceous chondrites, probably of type CI (https://en.wikipedia.org/wiki/Carbonaceous_chondrite#CI_group), and add ices of volatiles.
Gerald: I saw a mass ratio 8 parts to 2 and volume ratio 3 parts to 2 mentioned (cannot locate the reference, think it was DPS). That suggests a density of 8/3 for the rocks, which matches the composition you suggest.
Thanks, Kamal, for cross-checking!
Gerald: Sorry, I think my calculation is wrong, one has to take into account other features like porosity.
Kamal
Doesn’t porosity cancel out in this example?
Depends on which density is meant.
Interesting. But why the need to add ices of volatiles? And was Philae’s drill designed to penetrate this type of material?
The need to add ices of volatiles depends on the history of the boulders. If they haven’t been exposed to sufficient solar heat, it’s better to assume those ices, since evolving gases are detected near the comet. They may or may not originate from the boulders, too, besides the matrix.
Carbonaceous chondrites found on Earth contain several percent (up to more then 20%) of water (“CI chondrites typically contain a high proportion of water (up to 22%)”, https://en.wikipedia.org/wiki/Carbonaceous_chondrite#CI_group). That’s not a proof, that other volatiles need to be contained, but the Rosetta measurements don’t indicate pure water emissions, but a mix with other volatiles. So it appears more reasonable to assume other volatiles in the boulders, too. But, as said before, if those boulders have been heated by the Sun, supervolatiles may be depleted already, leading to a higher percentage of refractory material.
Philae’s drill was designed to penetrate some range of materials, mostly soft ones. The designed upper limit for compressive strength has been about 2.5 MPa.
(source: “MUPUS insertion device for the Rosetta mission”, Jerzy Grygorczuk, Marek Banaszkiewicz, Karol Seweryn, and Tilman Spohn)
This corresponds to about 25 bar pressure.
Ice has been determined to be around 73.8 bar (with large variability) at -9.4°C, see table I of this paper:
https://www.igsoc.org:8080/journal/19/81/igs_journal_vol19_issue081_pg213-223.pdf
The problem: Temperature has been measured to be near -170°C at the landing site of Philae.
“Probe discovers hard ice and measures temperature of minus 170 degrees Celsius on Comet 67P/Churyumov-Gerasimenko”
https://www.dlr.de/dlr/en/desktopdefault.aspx/tabid-10081/151_read-12189/#/gallery/17224
At these temperatures, water ice is a lot harder; no chance to be penetrated by the MUPUS hammer.
“…pure water ice at 100K has almost 4 times the dynamic compressive strength of ice at 258K…”
https://www.hou.usra.edu/meetings/ippw2014/pdf/8052.pdf
So, you don’t need silicate rock to get a problem with MUPUS. Cold, (almost) massive ice is more than sufficient.
The considered carbonaceous chondrites are rather soft (don’t know for sure, since they are expensive, not to waste for destructive experiments), but with frozen ices, who knows.
… meant frozen volatiles.
Grains larger than about 2 meters are called “boulders”, independent of their composition:
https://en.wikipedia.org/wiki/Grain_size
Fourth image appears to me as two high activity zones, which have progressively eroded and cleaned the surface. So, not sure if those large, fractured boulders have fallen, or just erosion remains.
The upper one show clues of radiality in the gas outflow.
https://blogs.esa.int/rosetta/files/2015/11/Rosetta_OSIRIS_Pajola_Figure1.jpg
Rest of the photos, including that boulder field at Hapi region, expressing same model preference 🙂
There is no other geology, where mathematicians have more to tell us.
That could be one of reasons for OSIRIS phantasmal presence.